Research Topics
Solar and stellar dynamos
Observations of Sunlike stars show various signs of magnetic activity at their surface. The level of magnetic activity is main tained by a mechanism, called the dynamo. The dynamo acts below the surface resulting in cyclic activity, which appears at various latitudes. Details of the dynamo process have yet to be clarified. Two competing theories attempt to pierce this unsolved issue: the flux transport theory suggests that the spots seen at the solar surface are actively participating in the dynamo mechanism, whereas the turbulent theory suggests that spots are only a signature of the underlying dynamo. With global numerical simulations, carried out at the institute’s cluster, a nonlinear model could be built for the flux transport theory which, for the first time, reproduces solar activity quantitatively. If such a nonlinear model correctly describes the magnetic activities of further younger and less massive stars, it would be a hint that stellar spots do indeed play an active role in the dynamo mechanism.
Solar and stellar differential rotation
The solar convection zone rotates differentially, i.e. the rotation period is shortest at the equator and longest at the poles. This pattern can be explained by the influence of the Coriolis force, which plays an essential role in the generation of magnetic fields. As such, the question of their rotational behaviour is of major importance to the study of the magnetic activity of stars. The differential rotation of stars can be measured by observing brightness fluctuations caused by spots on the star’s surface. While theoretical models have until recently predicted a solar-type differential rotation, in certain cases an opposite (“antisolar”) pattern has been found in numerical simulations. Simulations of angular momentum transport in rotating convection have shown that convective angular momentum transport for slowly rotating stars actually reverses its direction, resulting in an accelerated rotation of the polar caps.
Planet formation
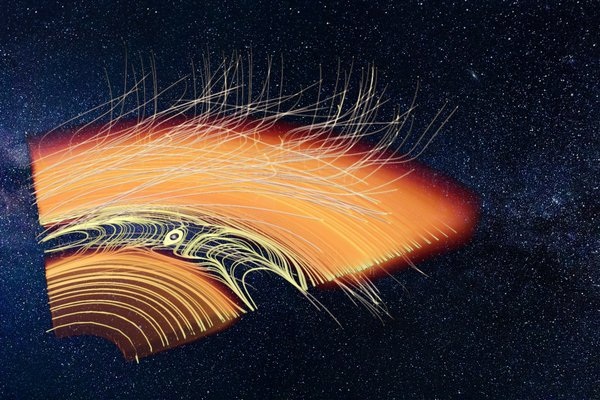
Protoplanetary gas disk with a gap opened by a planet. The visualization shows the gas density including flow lines (yellowish) and magnetic field lines (white).
Credit: AIP / O. GresselProtoplanetary disks accrete via magnetic stresses. When the effect of ambipolar diffusion is included, the planet-forming inner region develops a magnetocentrifugal disk wind. The world’s first global MHD simulations of this were performed using the AIP-developed NIRVANA code. Extended with radiative transfer and thermochemistry, the comprehensive framework is aimed at defining the standard in the field. Protoplanetary disks are of key interest to planet formation theory. Their dynamical, radiative, and thermal properties critically define the environment for embedded solids. The disks’ dynamics and structure in turn depend on the influence of magnetic fields. In an effort funded by the European Research Council (ERC), stateoftheart simulations were combined with comprehensive postprocessing to produce synthetic observations in the ALMA bands. The goal is to witness planet formation “in flagranti”.
[more]Interstellar chemistry and pre-stellar core formation
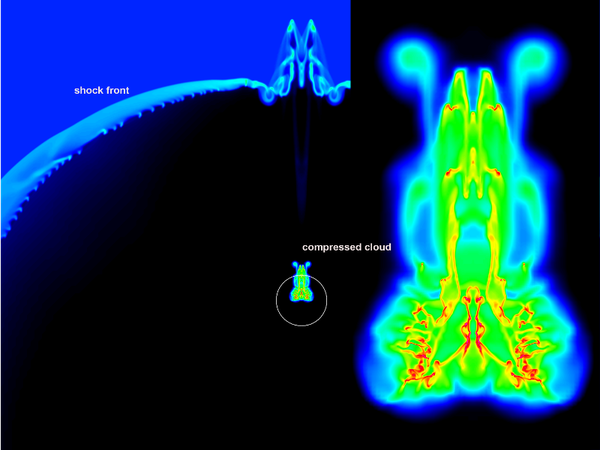
Supernova explosions produce strong shock waves that propa gate in the interstellar medium at high speed. If such a shock wave hits a molecular cloud, prestellar gas cores can be formed by compression. Realistic simulations of this interaction require the use of highly complex computer models which, in addition to magnetohydrodynamics on widely varying spatial scales, must also take into account chemothermal processes in the interstellar gasdust mixture. To enable such a model, the NIRVANA code was expanded to include ionisation and molecular chemistry. The temporal evolution of the chemical composition is of funda mental importance, since it regulates the strength of the associated cooling processes in the gas, influencing the dynamics. Simulations are used to investigate how the level of detail in the modelling of important chemo-thermal processes affects the formation of gas cores, and under which conditions such star precursors are formed in the first place.
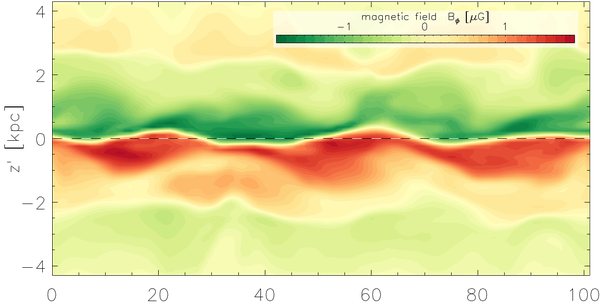
Magnetic field undulation resulting from the Parker instability in a hybrid Galactic dynamo simulation (i.e., evolving fluid dynamics alongside a mean-field dynamo model). Such undulation would not have shown up in a classical dynamo simulation and may explain (apparent) radial "reversals" of the magnetic field direction seen in the Milky-way but not in external galaxies.
Credit: AIP/O. GresselGalactic dynamos
Polarised synchrotron emission shows radio signatures of large-scale magnetic structures in the Milky Way and external galaxies. The origin of these magnetic fields can also be explained by a dynamo mechanism. Expensive numerical computations of the turbulent interstellar medium could directly simulate the long suspected dynamo. The turbulence properties of the interstellar medium relevant for the dynamo were determined. A global dynamical model of the magnetic field in the Milky Way was computed. In the inner part up to 10 kpc the kinematic dynamo dominates. With outwards decreasing star formation rate the magneto-rotational instability determines the magnetic field configuration.
Magnetic fields and the structure of the Sun
Physical models of the structure of the Sun and stars can reproduce the observed quantities with an impressive accuracy. Fusion in the core, various properties of the gas, and an approximation for heat transport by turbulence are the usual ingredients. Since some stars appear larger than predicted by the models, magnetic fields were suspected of being the missing element. Computations of magnetohydrodynamics (MHD) introduce mag netic fields into a stellar-structure model and consider their effect on the heat transport. The distribution of magnetic fields inside the Sun comes from dynamo models, reproducing the 11year sunspot cycle. The effect of magnetic fields causes strong fields measuring over one tesla to change the solar radius by a few parts per million. Observable radius changes need to be ten times larger. The surface temperature, however, shows a variation of about half a degree, which may be measurable.
Magnetospheres of massive stars
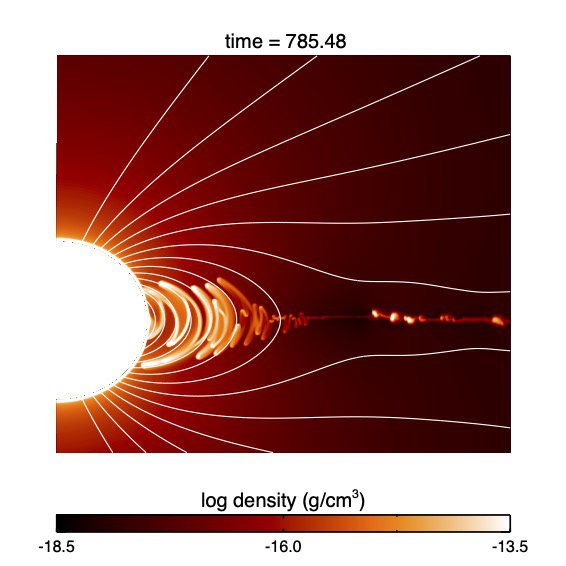
Gas distribution and magnetic field geometry around a massive star. The colour contours show mass density, the white lines the magnetic field.
Credit: AIP / M. KuekerMassive stars are very luminous and lose large amounts of gas through radiation-driven winds. These winds carry away up to one billion times as much mass as the solar wind and reach gas velocities of one percent of the speed of light. Large-scale surface magnetic fields with field strengths exceeding one kilogauss must have a profound impact on the stellar winds. Far away from the star the magnetic field follows the gas flow and the field lines are open. Near the stellar surface, however, closed field loops can exist and gas be trapped. Numerical simulations of the interaction of wind and magnetic field show that an initially dipolar magnetic field opens up at large distances from the star. Field lines originating near the equator, however, never reach large distances from the star and remain closed. Gas therefore gets trapped at low latitudes, where it falls back to the star along the magnetic field lines. Outside the closed field line area, a current layer forms in the equatorial plane, where magnetic fields of opposite polarities cancel each other out and a gas disk forms. In the open field line region the gas escapes into the interstellar medium.
Advanced Turbulence Diagnostics
Essentially all astrophysical fluids are found in a state of chaotic, turbulent motion. Owing to the immense dynamic range of scales, modelling the evolution of celestial objects as a whole thus demands the development of effective “sub-grid-scale“ models. These describe fluid motions on unresolved scales and, in particular, their effect on the large-scale dynamics (e.g., via angular momentum transport, diffusion and mixing of chemical contaminants, induction of dynamo fields, etc.). The MHD group is leading in the development and application of the so-called test-field approach used for reliably sensing mean-field effects in turbulent flows. By introducing spatially and temporally modulated mean fields, we have recently generalised our approach to the case of a novel non-local and non-instantaneous closure relation, accounting for and extended “domain of dependence” in space, and “memory effects” in time.